1. Introduction
In the field of wind power generation, the reliable operation of wind turbine gearboxes is crucial for the efficient conversion of wind energy into electrical energy. Sliding bearings, with their excellent performance and relatively low maintenance costs, are becoming the preferred choice for gearbox bearings, especially as wind turbines increase in power and the radial dimensions of rolling bearings lead to higher failure rates. However, the manufacturing process of sliding bearings, particularly the centrifugal casting technique used to create the bronze sliding layer on the surface, is not without its challenges.
Centrifugal casting, while an effective method for achieving metal metallurgical fusion, can result in defects such as debonding, porosity, and looseness at the bimetallic interface of the workpiece. These defects, especially debonding, can compromise the integrity of the bearing structure and pose a significant threat to the operation of the gearbox. Given the high cost of downtime for maintenance due to the large size and elevated position of wind turbines, non-destructive testing (NDT) of the bimetallic interface of the workpiece is of utmost importance to ensure the reliability of the bearing.
Ultrasonic testing has emerged as a leading NDT method for detecting internal defects in metal materials due to its ease of use, high sensitivity, and wide detection range. By leveraging the principles of ultrasonic wave propagation and interaction with materials, it offers a means to identify and characterize defects that may not be visible or detectable by other means. This article focuses on the application of ultrasonic testing to detect debonding defects in the sliding bearings of wind turbine gearboxes, aiming to provide a comprehensive understanding of the techniques and methodologies involved.
2. Wind Turbine Sliding Bearing Debonding Defects
2.1 Structure and Function of Wind Turbine Sliding Bearings
Wind turbine sliding bearings consist of an outer copper alloy layer and an inner steel substrate. The copper alloy layer, typically a bronze alloy, serves as the sliding surface, providing excellent wear resistance and compatibility with the lubricant. The steel substrate, on the other hand, offers the necessary strength and rigidity to support the loads imposed during operation. This combination of materials is designed to withstand the harsh operating conditions of wind turbine gearboxes, including high loads, low speeds, and potential misalignments.
The bronze sliding layer is crucial for ensuring smooth and efficient operation of the bearing. It reduces friction and wear between the moving parts, thereby increasing the service life of the bearing and minimizing the need for frequent maintenance. Additionally, the self-lubricating properties of the bronze layer contribute to the overall reliability of the bearing system, even in situations where the lubrication supply may be intermittent or insufficient.
2.2 Causes and Consequences of Debonding Defects
Debonding defects at the interface between the bronze sliding layer and the steel substrate can occur due to various factors related to the centrifugal casting process. Poor liquidity of the liquid metal during casting can prevent proper adhesion between the two layers, leading to incomplete bonding. Temperature non-uniformity within the casting mold can cause differential contraction rates, creating internal stresses that can result in the separation of the layers. The differences in the physical and chemical properties of the copper and steel materials, such as their coefficients of thermal expansion and Young’s moduli, can also contribute to the formation of debonding defects.
The presence of debonding defects has severe consequences for the performance and lifespan of the sliding bearing. These defects increase the local deformation of the bearing, leading to uneven stress distribution and accelerated wear. In severe cases, the bronze sliding layer may detach completely from the steel substrate, causing the bearing to fail catastrophically. Such a failure can lead to costly downtime of the wind turbine, as well as potential damage to other components of the gearbox and drivetrain.
3. Sound Field Simulation Theory
3.1 Wave Equation and Finite Element Method
The propagation of ultrasonic waves in isotropic media is governed by the wave equation, which relates the stress and displacement within the medium. By neglecting volume forces such as gravity, the wave equation can be expressed as , where is the Hamiltonian operator, is the solid density, is the displacement vector, and and are the Lamé constants.
The finite element method (FEM) is a powerful numerical technique for simulating ultrasonic testing. It involves dividing the computational region into a mesh of elements with specific shapes and sizes. By constructing a displacement function matrix for each element and applying the principle of virtual work, the FEM can transform the forces acting on the mesh into nodal forces. This allows the derivation of the system’s motion equation, which, in the absence of damping, can be simplified to , where and are the nodal acceleration and velocity vectors, respectively, and , , and are the system mass matrix, stiffness matrix, and nodal load vector.
3.2 Reflection and Transmission of Ultrasonic Waves at Interfaces
When ultrasonic waves encounter an interface between different media, they undergo reflection and transmission. The reflection and transmission coefficients depend on the relative acoustic impedances of the two media. For normal incidence, the acoustic pressure reflection coefficient and transmission coefficient are given by and , respectively, where and are the acoustic impedances of the two media on either side of the interface.
In the case of a copper-steel interface, the relatively small difference in acoustic impedance between copper and steel results in a small reflection coefficient, allowing most of the ultrasonic wave to transmit into the steel substrate. However, when a debonding defect is present, the interface is effectively replaced by an air layer, which has a much lower acoustic impedance than either copper or steel. This causes a significant increase in the reflection coefficient, leading to the generation of a strong defect wave that can be detected and used to identify the presence and location of the defect.
3.3 Simulation Model and Boundary Conditions
To simulate the ultrasonic testing of a copper-steel bimetallic casting workpiece, a two-dimensional (2D) model is often used due to its computational efficiency compared to a three-dimensional (3D) model. The model consists of a copper outer layer and a steel inner layer, with an elastic thin layer introduced at the interface to simulate the debonding defect. The dimensions of the model are chosen to represent a small section of the actual workpiece, with the copper layer having a thickness of 6 mm and the steel layer a thickness of 15 mm.
The excitation source is modeled as a 10 MHz sinusoidal pulse modulated by a Hanning window to reduce high-frequency interference and spectral leakage. The boundary conditions are carefully set to mimic the actual testing conditions. The interface between the layers is set as a free boundary to allow for the simulation of debonding, while the left and right boundaries of the solid and the excitation source are set as low-reflection boundaries to suppress unwanted echoes. The elastic thin layer representing the debonding defect is modeled using spring elements to couple the vibrations of the adjacent solid layers.
4. Numerical Simulation Results
4.1 Propagation of Ultrasonic Waves in Defect-Free and Defective Models
In the defect-free model, when the ultrasonic wave reaches the copper-steel interface, most of the wave energy is transmitted into the steel substrate, with only a small portion being reflected back. This is consistent with the relatively small difference in acoustic impedance between copper and steel. The transmitted wave continues to propagate through the steel layer until it reaches the bottom surface, where it is reflected back to the transducer, generating the bottom wave.
In the presence of a debonding defect, the ultrasonic wave is almost completely reflected at the interface between the copper and the elastic thin layer (simulating air). This results in a strong defect wave being generated, which travels back to the transducer. The remaining wave energy that is transmitted through the defect continues to propagate in the steel layer, but with a reduced amplitude due to the energy loss at the defect.
4.2 A-Scan Echo Signals and Their Relationship with Defect Sizes
The A-scan echo signals obtained from the simulation show distinct characteristics for different defect sizes. In the defect-free case, two main peaks are observed, corresponding to the interface echo and the bottom wave. The time difference between these peaks can be used to calculate the thickness of the copper-steel layer.
When a defect is present, an additional peak corresponding to the defect wave appears. The amplitude of the defect wave increases with increasing defect size, while the amplitude of the bottom wave decreases. This is because as the defect size grows, more of the ultrasonic wave energy is reflected back at the defect, leaving less energy to reach the bottom surface. The relationship between the amplitude of the defect wave and the defect size is approximately linear for defect sizes between 2 mm and 8 mm. For defect sizes smaller than 2 mm, the presence of the initial interface echo and the relatively small defect size result in a less significant change in the defect wave amplitude. For defect sizes larger than 8 mm, the defect wave amplitude reaches a maximum as the defect size exceeds the diameter of the transducer, and further increases in defect size do not significantly affect the amplitude.
4.3 C-Scan Imaging of Defects
C-scan imaging provides a visual representation of the distribution and size of defects within the workpiece. By scanning the ultrasonic transducer across the surface of the workpiece and recording the amplitude of the defect wave at each point, a 2D image can be constructed. In the simulation, the C-scan image clearly shows the location and relative size of the debonding defect. The defect appears as a distinct region with a higher amplitude of the defect wave compared to the surrounding defect-free areas.
The C-scan imaging technique can be used to quickly identify the presence and extent of defects in the sliding bearing, providing valuable information for quality control and maintenance decisions. By comparing the C-scan images with the actual dimensions of the workpiece, the accuracy of the ultrasonic testing method can be evaluated.
5. Experimental Validation
5.1 Sample Preparation and Experimental Equipment
To validate the simulation results, samples with artificial debonding defects were prepared. Flat-bottom holes with diameters of 2, 4, 6, 8, and 10 mm were machined at the copper-steel interface of the sliding bearing samples. The depth of these holes was set to 15 mm to simulate the debonding defects.
Two types of experimental setups were used for the ultrasonic testing: contact ultrasonic testing and water immersion ultrasonic testing. The contact ultrasonic testing setup consisted of an Olympus A552S-SM contact probe (10 MHz), a TBS2000B oscilloscope, and a DPR300 ultrasonic pulse transmitter/receiver. The water immersion ultrasonic testing setup used a custom-made water immersion point-focusing probe (15 MHz) from Shanghai Jiuheng Instrument Co., Ltd., and an Anno 450 robotic arm for scanning.
5.2 Contact Ultrasonic Testing Results
During the contact ultrasonic testing, the ultrasonic waves were transmitted into the sample through the contact probe, and the reflected echoes were received and recorded. The obtained echo signals showed the presence of multiple peaks, including the initial wave, interface echo, defect wave, and bottom wave. The amplitudes and time intervals between these peaks were analyzed to determine the characteristics of the defects.
The experimental results were consistent with the simulation in terms of the general trends. As the defect size increased, the amplitude of the defect wave increased, and the amplitude of the bottom wave decreased. However, the experimental echo signals also contained more noise compared to the simulation results. This was mainly due to the scattering of ultrasonic waves by the coarse grains in the material, which was not considered in the simulation model.
5.3 Water Immersion Ultrasonic Testing Results
In the water immersion ultrasonic testing, the sample was immersed in water, and the point-focusing probe was used to scan the surface of the sample. The ultrasonic waves were transmitted through the water and into the sample, and the reflected waves were detected and used to generate C-scan images.
The C-scan images obtained from the experiment showed the location and approximate size of the artificial defects. The detected defect sizes were generally in good agreement with the actual defect sizes, although some discrepancies were observed. These discrepancies were mainly attributed to the inaccuracies in the positioning of the robotic arm during the scanning process and the variation in the scanning speed near the right limit of the arm’s motion.
6. Comparison and Analysis of Simulation and Experimental Results
6.1 Agreement and Discrepancies in Defect Wave and Bottom Wave Amplitudes
The comparison between the simulation and experimental results for the amplitudes of the defect wave and the bottom wave showed a high degree of agreement in terms of the overall trends. Both the simulation and experiment demonstrated that the amplitude of the defect wave increased with increasing defect size, while the amplitude of the bottom wave decreased. This linear relationship between the amplitudes and the defect sizes provided a reliable basis for the quantitative characterization of the debonding defects.
However, some differences were also noted. The experimental defect wave amplitudes were generally slightly lower than the simulated values, especially for larger defect sizes. This could be attributed to the energy losses in the actual testing process due to factors such as the coupling between the probe and the sample surface and the attenuation of the ultrasonic waves in the material. The experimental bottom wave amplitudes also showed more significant fluctuations compared to the simulation, which was mainly due to the presence of noise and the non-uniform microstructure of the actual samples.
6.2 Evaluation of C-Scan Imaging Accuracy
The C-scan imaging results from the experiment were compared with the actual defect sizes and positions to evaluate the accuracy of the ultrasonic testing method. The overall agreement between the detected and actual defect locations was satisfactory, although some deviations were observed, particularly for larger defect sizes. The inaccuracies in the C-scan imaging were mainly caused by the limitations of the scanning equipment and the difficulties in precisely positioning the probe over the defects.
Despite these discrepancies, the C-scan imaging technique still provided valuable information about the distribution and relative size of the defects, which could be used for preliminary defect assessment and quality control purposes.
6.3 Significance of the Research Results and Their Implications for Practical Applications
The results of this study demonstrated the effectiveness of using ultrasonic testing, both in simulation and experiment, to detect and characterize debonding defects in wind turbine gearbox sliding bearings. The ability to accurately identify the presence and size of defects is crucial for ensuring the reliability and safety of wind turbine operation.
In practical applications, the findings of this research can be used to develop more reliable and efficient inspection procedures for sliding bearings. By implementing ultrasonic testing as a routine inspection method, potential defects can be detected early, allowing for timely maintenance or replacement of the bearings. This can significantly reduce the risk of unexpected failures and costly downtime of wind turbines.
7. Conclusion and Future Research Directions
7.1 Summary of the Research Findings
In this study, a comprehensive investigation was conducted on the ultrasonic detection of debonding defects in wind turbine gearbox sliding bearings. The following key findings were obtained:
- The amplitude of the defect wave in the A-scan echo signal is approximately linearly related to the defect size, with an increasing trend. Conversely, the amplitude of the bottom wave shows a linear negative correlation with the defect size.
- The simulation results using Comsol Multiphysics software are in good agreement with the experimental results, validating the effectiveness of the simulation model in predicting the behavior of ultrasonic waves in the presence…
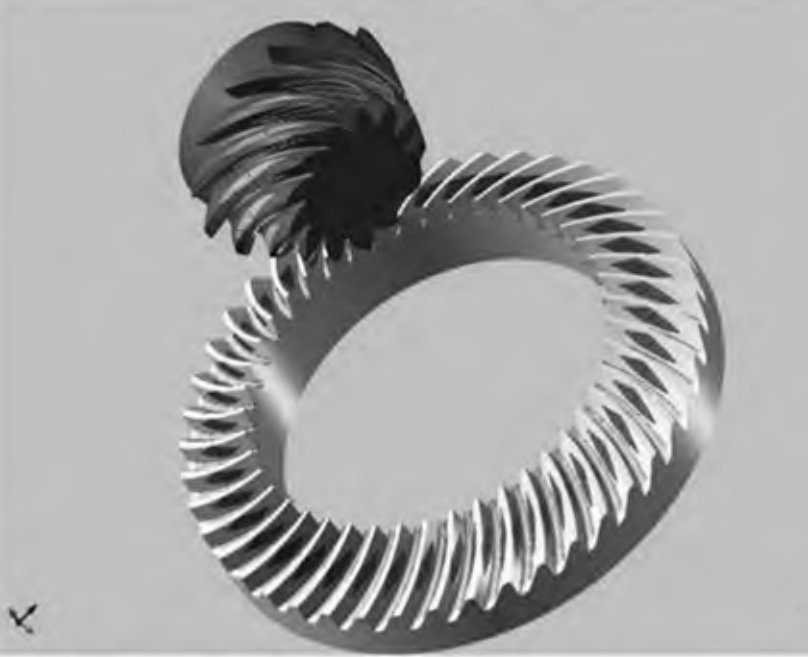